#particle detectors
Explore tagged Tumblr posts
Text
Giant particle detectors for neutrino project - Technology Org
New Post has been published on https://thedigitalinsider.com/giant-particle-detectors-for-neutrino-project-technology-org/
Giant particle detectors for neutrino project - Technology Org
With excavation work complete at the site where four gigantic particle detectors for the international Deep Underground Neutrino Experiment (DUNE) will be installed, scientists are preparing to begin construction on first detector. Part of that work occurs at The University of Texas at Arlington.
Jaehoon Yu, UTA professor of physics, stands on an elevated platform inside the DUNE field cage prototype
Located a mile below the surface at the Sanford Underground Research Laboratory in Lead, South Dakota, the three colossal caverns serve as the core of a new research facility that spans an underground area about the size of eight soccer fields.
Hosted by the U.S. Department of Energy’s Fermi National Accelerator Laboratory (Fermilab), DUNE scientists will study the behavior of mysterious particles known as neutrinos to solve some of the biggest questions about the universe. These include why the universe is composed of matter, how an exploding star creates a black hole and if neutrinos are connected to dark matter or other undiscovered particles.
Jaehoon Yu, professor of physics, and Jonathan Asaadi, associate professor of physics, are leading UTA’s involvement with the project.
“The actual excavation took only a year, which is amazing,” Yu said. “It’s great that the excavation work is finished, and preparations can now be made for the installation of the detectors. This is an exciting time.”
The caverns provide space for four large neutrino detectors—each one about the size of a seven-story building. The detectors will be filled with liquid argon and record the rare interaction of neutrinos with the transparent liquid.
With DUNE, scientists will look for neutrinos from exploding stars and examine the behavior of a beam of neutrinos produced at Fermilab, located near Chicago, about 800 miles east of the underground caverns. The beam, produced by the world’s most intense neutrino source, will travel straight through earth and rock from Fermilab to the DUNE detectors in South Dakota. No tunnel is necessary for its path.
UTA’s Department of Physics has been involved with the DUNE project since its earliest stages. In January 2016, Yu organized a four-day international planning conference at UTA.
UTA physicists will now build portions of the first two detectors to be installed at the South Dakota site. Specifically, they will construct modules of the field cage—100 modules for the first detector and all 200 of the modules for the second detector. The work will take place in the Chemistry and Physics Building.
“We’re going to need to recruit a lot of students to help with this work,” Yu said. “We need to bring them in as freshmen and sophomores so we can train them, and they can be with the project as long as possible, including for the installation.”
Yu will lead UTA’s efforts for construction of parts for the “far” detector in South Dakota. Asaadi is working on portions of the “near” detector at Fermilab.
The DUNE collaboration includes more than 1,400 scientists and engineers from over 200 institutions in 36 countries.
Source: University of Texas at Arlington
You can offer your link to a page which is relevant to the topic of this post.
#amazing#argon#Behavior#black hole#Building#chemistry#Collaboration#conference#construction#Dark#dark matter#detector#earth#energy#engineers#Fermi#Fundamental physics news#how#interaction#it#Link#liquid#matter#neutrino#neutrinos#One#Other#particle#particle detectors#particles
0 notes
Text
Particle Detectors
Introduction Particle detectors are instruments designed for measuring and identifying particles, such as those produced by nuclear decay, cosmic radiation, or reactions in a particle accelerator. Detectors measure and record physical quantities such as energy, momentum, spin, charge, etc., of the particles. Basic Principles The operational principle of particle detectors is based on the…
View On WordPress
0 notes
Link
For your special NORDVPN discount and risk free 30 Day Money back guarantee, visit www.nordvpn.com/stuartgary and use the code STUARTGARY at checkout. Get your online privacy under control with the best in the business. The Space, Astronomy & Science Podcast. SpaceTime Series 27 Episode 33 *The Black Hole that Ripped Apart a Star In a cosmic display of destruction, astronomers have witnessed the closest recorded incident of a star being devoured by a supermassive black hole. The event, spotted in galaxy NGC 3799, was observed in visible light, offering unprecedented insights into these violent phenomena. The tidal disruption event, now cataloged as Assassin 23 BD, emitted less energy and faded more rapidly than typical cases, placing it in a unique classification and suggesting such occurrences may be more common than once thought. *Hunting New Physics in Neutron Star Wrecks The cataclysmic mergers of neutron stars could be hiding secrets of new physics, with potential clues about the enigmatic dark matter. The historic GW170817 collision, which sent ripples through space-time, has now been used to constrain theories on axion-like particles. These elusive entities could be part of the dark matter puzzle, and their traces might be detectable in future neutron star mergers, offering a new window into the fundamental workings of the universe. *Guardian Gas Giants and Their Terrestrial Charges A new study suggests that Earth-like planets often come with Jupiter-like protectors. Simulations of planetary systems show that "dry" super-Earths and distant "cold" Jupiters frequently coexist, with the gas giants acting as cosmic shields. This relationship hints at a formation pattern where massive protoplanetary disks give rise to both rocky worlds and their gaseous sentinels, providing a glimpse into the architecture of alien solar systems. *China's Moonwalk Ambitions by 2030 China has set its sights on the Moon, with plans to land taikonauts on the lunar surface before the end of the decade. The ambitious project involves the development of the Long March 10 rocket, a new spacecraft, and a lunar lander. In a two-launch scenario, the vehicles will rendezvous in lunar orbit, setting the stage for China's historic manned moon landing and furthering their collaboration with Russia on a lunar base. Plus, we examine the latest space news, delve into the science of extreme heat and preterm births, and explore the mystery of microplastics in human arteries. For more SpaceTime and to support the show, visit our website at https://spacetimewithstuartgary.com where you can access our universal listen link, find show notes, and learn how to become a patron. Listen to SpaceTime on your favorite podcast app with our universal listen link: https://spacetimewithstuartgary.com/listen and access show links via https://linktr.ee/biteszHQ Support the show: https://www.spreaker.com/podcast/spacetime-with-stuart-gary--2458531/support For more space and astronomy podcasts, visit our HQ at https://bitesz.com
#axion-like#black#collision#dark#detector#disruption#event#gamma#gravitational#hole#ligo#matter#neutron#particles#ray#star#supermassive#tidal#virgo#waves
2 notes
·
View notes
Text
not Star Trek: Strange New Worlds getting a very basic fact about the radioactive decay of tritium wrong
#let’s find a cold fusion reactor by walking around trying to find tritium#beta particles travel a few meters in air *at best*#they would never make it through any kind of containment structure#they can’t make it through skin! which is WHY it can be put into watches without unnecessarily exposing you to ionizing radiation#if they stole a neutron detector from a university I’d buy that#writing plausible treknobabble is hard! I know! but they can do better than this#star trek#star trek strange new worlds
5 notes
·
View notes
Text
Hypernuclei flow, enjoy!
7 notes
·
View notes
Photo
Always fascinating ⭐

8K notes
·
View notes
Text
For example, on October 15, 1991, the Fly's Eye cosmic ray detector, in the Utah desert, measured a particle streaking across the sky with an energy equivalent to 30 billion proton masses. That's almost as much energy in a single subatomic particle as in a Mariano Rivera fastball, and is about 100 million times the size of the particle energies that will be produced by the Large Hadron Collider.⁶
6. From the viewpoint of energetics, therefore, cosmic rays provide a naturally occurring accelerator that is far more powerful than any we have or will construct in the foreseeable future. The drawback is that although the particles in cosmic rays can have extremely high energies, we have no control over what slams into what – when it comes to cosmic ray collisions, we are passive observers. Furthermore, the number of cosmic ray particles with a given energy drops quickly as the energy level increases. While about 10 billion cosmic ray particles with an energy equivalent to the mass of a proton (about one-thousandth of the design capacity of the Large Hadron Collider) strike each square kilometer of earth's surface every second (and quite a few pass through your body every second as well), only about one of the most energetic particles (about 100 billion times the mass of a proton) would strike a given square kilometer of earth's surface each century. Finally, accelerators can slam particles together by making them move quickly, in opposite directions, thereby creating a large center of mass energy. Cosmic ray particles, by contrast, slam into the relatively slow moving particles in the atmosphere. Nevertheless, these drawbacks are not insurmountable. Over the course of many decades, experimenters have learned quite a lot from studying the more plentiful, lower-energy cosmic ray data, and, to deal with the paucity of high-energy collisions, experimenters have built huge arrays of detectors to catch as many particles as possible.
"The Fabric of the Cosmos" - Brian Greene
#book quotes#the fabric of the cosmos#brian greene#nonfiction#october 15#october#90s#1990s#fly's eye#cosmic rays#utah#desert#particle#energy#subatomic particles#mariano rivera#fastball#large hadron collider#lhc#detection#detectors
0 notes
Text
Whenever the detector is on – again, even if the choice to turn it on is delayed until long after a given photon has passed through the beam splitter – the photon acts fully like a particle.
"The Fabric of the Cosmos" - Brian Greene
#book quote#the fabric of the cosmos#brian greene#nonfiction#detector#delay#photon#laser beam#particle
0 notes
Text
Okay so a guy in my solid state physics class was telling us about this muon scanning startup he worked at, GScan, and I'm going insane. I don't work there and I have no stake in the company, financial or otherwise, I just need to tell you about it.

Muons are short-lived subatomic particles, same charge as an electron but ~200 times more massive. On Earth, they're produced by cosmic rays colliding with the upper atmosphere, and they hit the ground at a rate of about ten thousand per minute per square meter.
They're moving extremely fast at ground level, like 0.99 c. So they careen right through matter, deflecting only very slightly around heavy atomic nuclei – they'll penetrate like a hundred meters into solid rock.
What do you do with this continuous shower of deep-penetrating charged particles, constantly blanketing every square inch of the Earth's surface?
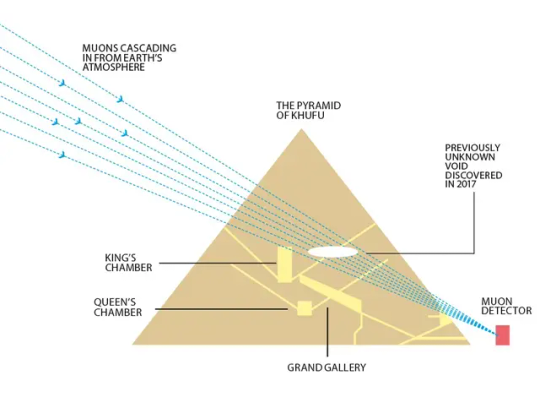
(source)
The classic thing is use them to image the inside of massive structures, like we use x-rays to look inside living tissue – except instead of generating them yourself, you just use atmospheric muons. Muon archeology is a whole thing, they've used it to find hidden chambers in pyramids and stuff. Neat!
But this one Estonian company is doing some crazy bullshit and I love it.
Sandwich anything between a pair of portable muon detectors and get full 3D imaging of the interior, with sub-millimeter accuracy, by tracking the minute deflection of muons between them. Samples that are WAY too thick for x-rays, made of literally anything. Just put some muon detectors on some two by fours in a warehouse and call it a day.
You can just. Image anything??? Anything you want?? Completely passively!! Just detectors! No particle source! Put them anywhere. The detectors themselves are a mature technology, the company's tech is in the algorithms they use to get this level of spatial and elemental resolution.
You can detect failures inside cable-reinforced concrete bridges without cutting open the bridges.
Decommissioned Soviet nuclear submarine filled with concrete, with no drawings or documentation, that may or may not have spent fuel canisters in it? And you need to cut it up for storage? Just look at the muons.
One of the wackiest ideas is to put one detector under your bed and one on the ceiling, so you get a full 3D scan of your body every night, passively. I want one.
7K notes
·
View notes
Text
Conceptual Design for a Neutrino Power Transmission System
Overview
Neutrinos could potentially be used to send electricity over long distances without the need for high-voltage direct current (HVDC) lines. Neutrinos have the unique property of being able to pass through matter without interacting with it, which makes them ideal for transmitting energy over long distances without significant energy loss. This property allows neutrinos to be used as a medium for energy transmission, potentially replacing HVDC lines in certain applications.
So the goal is to create a neutrino-based power transmission system capable of sending and receiving a beam of neutrinos that carry a few MW of power across a short distance. This setup will include a neutrino beam generator (transmitter), a travel medium, and a neutrino detector (receiver) that can convert the neutrinos' kinetic energy into electrical power.
1. Neutrino Beam Generator (Transmitter)
Particle Accelerator: At the heart of the neutrino beam generator will be a particle accelerator. This accelerator will increase the energy of protons before colliding them with a target to produce pions and kaons, which then decay into neutrinos. A compact linear accelerator or a small synchrotron could be used for this purpose.
Target Material: The protons accelerated by the particle accelerator will strike a dense material target (like tungsten or graphite) to create a shower of pions and kaons.
Decay Tunnel: After production, these particles will travel through a decay tunnel where they decay into neutrinos. This tunnel needs to be under vacuum or filled with inert gas to minimize interactions before decay.
Focusing Horns: Magnetic horns will be used to focus the charged pions and kaons before they decay, enhancing the neutrino beam's intensity and directionality.
Energy and Beam Intensity: To achieve a few MW of power, the system will need to operate at several gigaelectronvolts (GeV) with a proton beam current of a few tens of milliamperes.
2. Travel Medium
Direct Line of Sight: Neutrinos can travel through the Earth with negligible absorption or scattering, but for initial tests, a direct line of sight through air or vacuum could be used to simplify detection.
Distance: The initial setup could span a distance from a few hundred meters to a few kilometers, allowing for measurable neutrino interactions without requiring excessively large infrastructure.
3. Neutrino Detector (Receiver)
Detector Medium: A large volume of water or liquid scintillator will be used as the detecting medium. Neutrinos interacting with the medium produce a charged particle that can then be detected via Cherenkov radiation or scintillation light.
Photodetectors: Photomultiplier tubes (PMTs) or Silicon Photomultipliers (SiPMs) will be arranged around the detector medium to capture the light signals generated by neutrino interactions.
Energy Conversion: The kinetic energy of particles produced in neutrino interactions will be converted into heat. This heat can then be used in a traditional heat-to-electricity conversion system (like a steam turbine or thermoelectric generators).
Shielding and Background Reduction: To improve the signal-to-noise ratio, the detector will be shielded with lead or water to reduce background radiation. A veto system may also be employed to distinguish neutrino events from other particle interactions.
4. Control and Data Acquisition
Synchronization: Precise timing and synchronization between the accelerator and the detector will be crucial to identify and correlate neutrino events.
Data Acquisition System: A high-speed data acquisition system will collect data from the photodetectors, processing and recording the timing and energy of detected events.
Hypothetical Power Calculation
To estimate the power that could be transmitted:
Neutrino Flux: Let the number of neutrinos per second be ( N_\nu ), and each neutrino carries an average energy ( E_\nu ).
Neutrino Interaction Rate: Only a tiny fraction (( \sigma )) of neutrinos will interact with the detector material. For a detector with ( N_d ) target nuclei, the interaction rate ( R ) is ( R = N_\nu \sigma N_d ).
Power Conversion: If each interaction deposits energy ( E_d ) into the detector, the power ( P ) is ( P = R \times E_d ).
For a beam of ( 10^{15} ) neutrinos per second (a feasible rate for a small accelerator) each with ( E_\nu = 1 ) GeV, and assuming an interaction cross-section ( \sigma \approx 10^{-38} ) cm(^2), a detector with ( N_d = 10^{30} ) (corresponding to about 10 kilotons of water), and ( E_d = E_\nu ) (for simplicity in this hypothetical scenario), the power is:
[ P = 10
^{15} \times 10^{-38} \times 10^{30} \times 1 \text{ GeV} ]
[ P = 10^{7} \times 1 \text{ GeV} ]
Converting GeV to joules (1 GeV ≈ (1.6 \times 10^{-10}) J):
[ P = 10^{7} \times 1.6 \times 10^{-10} \text{ J/s} ]
[ P = 1.6 \text{ MW} ]
Thus, under these very optimistic and idealized conditions, the setup could theoretically transmit about 1.6 MW of power. However, this is an idealized maximum, and actual performance would likely be significantly lower due to various inefficiencies and losses.
Detailed Steps to Implement the Conceptual Design
Step 1: Building the Neutrino Beam Generator
Accelerator Design:
Choose a compact linear accelerator or a small synchrotron capable of accelerating protons to the required energy (several GeV).
Design the beamline with the necessary magnetic optics to focus and direct the proton beam.
Target Station:
Construct a target station with a high-density tungsten or graphite target to maximize pion and kaon production.
Implement a cooling system to manage the heat generated by the high-intensity proton beam.
Decay Tunnel:
Design and construct a decay tunnel, optimizing its length to maximize the decay of pions and kaons into neutrinos.
Include magnetic focusing horns to shape and direct the emerging neutrino beam.
Safety and Controls:
Develop a control system to synchronize the operation of the accelerator and monitor the beam's properties.
Implement safety systems to manage radiation and operational risks.
Step 2: Setting Up the Neutrino Detector
Detector Medium:
Select a large volume of water or liquid scintillator. For a few MW of transmitted power, consider a detector size of around 10 kilotons, similar to large neutrino detectors in current experiments.
Place the detector underground or in a well-shielded facility to reduce cosmic ray backgrounds.
Photodetectors:
Install thousands of photomultiplier tubes (PMTs) or Silicon Photomultipliers (SiPMs) around the detector to capture light from neutrino interactions.
Optimize the arrangement of these sensors to maximize coverage and detection efficiency.
Energy Conversion System:
Design a system to convert the kinetic energy from particle reactions into heat.
Couple this heat to a heat exchanger and use it to drive a turbine or other electricity-generating device.
Data Acquisition and Processing:
Implement a high-speed data acquisition system to record signals from the photodetectors.
Develop software to analyze the timing and energy of events, distinguishing neutrino interactions from background noise.
Step 3: Integration and Testing
Integration:
Carefully align the neutrino beam generator with the detector over the chosen distance.
Test the proton beam operation, target interaction, and neutrino production phases individually before full operation.
Calibration:
Use calibration sources and possibly a low-intensity neutrino source to calibrate the detector.
Adjust the photodetector and data acquisition settings to optimize signal detection and reduce noise.
Full System Test:
Begin with low-intensity beams to ensure the system's stability and operational safety.
Gradually increase the beam intensity, monitoring the detector's response and the power output.
Operational Refinement:
Refine the beam focusing and detector sensitivity based on initial tests.
Implement iterative improvements to increase the system's efficiency and power output.
Challenges and Feasibility
While the theoretical framework suggests that a few MW of power could be transmitted via neutrinos, several significant challenges would need to be addressed to make such a system feasible:
Interaction Rates: The extremely low interaction rate of neutrinos means that even with a high-intensity beam and a large detector, only a tiny fraction of the neutrinos will be detected and contribute to power generation.
Technological Limits: The current state of particle accelerator and neutrino detection technology would make it difficult to achieve the necessary beam intensity and detection efficiency required for MW-level power transmission.
Cost and Infrastructure: The cost of building and operating such a system would be enormous, likely many orders of magnitude greater than existing power transmission systems.
Efficiency: Converting the kinetic energy of particles produced in neutrino interactions to electrical energy with high efficiency is a significant technical challenge.
Scalability: Scaling this setup to practical applications would require even more significant advancements in technology and reductions
in cost.
Detailed Analysis of Efficiency and Cost
Even in an ideal scenario where technological barriers are overcome, the efficiency of converting neutrino interactions into usable power is a critical factor. Here’s a deeper look into the efficiency and cost aspects:
Efficiency Analysis
Neutrino Detection Efficiency: Current neutrino detectors have very low efficiency due to the small cross-section of neutrino interactions. To improve this, advanced materials or innovative detection techniques would be required. For instance, using superfluid helium or advanced photodetectors could potentially increase interaction rates and energy conversion efficiency.
Energy Conversion Efficiency: The process of converting the kinetic energy from particle reactions into usable electrical energy currently has many stages of loss. Thermal systems, like steam turbines, typically have efficiencies of 30-40%. To enhance this, direct energy conversion methods, such as thermoelectric generators or direct kinetic-to-electric conversion, need development but are still far from achieving high efficiency at the scale required.
Overall System Efficiency: Combining the neutrino interaction efficiency and the energy conversion efficiency, the overall system efficiency could be extremely low. For neutrino power transmission to be comparable to current technologies, these efficiencies need to be boosted by several orders of magnitude.
Cost Considerations
Capital Costs: The initial costs include building the particle accelerator, target station, decay tunnel, focusing system, and the neutrino detector. Each of these components is expensive, with costs potentially running into billions of dollars for a setup that could aim to transmit a few MW of power.
Operational Costs: The operational costs include the energy to run the accelerator and the maintenance of the entire system. Given the high-energy particles involved and the precision technology required, these costs would be significantly higher than those for traditional power transmission methods.
Cost-Effectiveness: To determine the cost-effectiveness, compare the total cost per unit of power transmitted with that of HVDC systems. Currently, HVDC transmission costs are about $1-2 million per mile for the infrastructure, plus additional costs for power losses over distance. In contrast, a neutrino-based system would have negligible losses over distance, but the infrastructure costs would dwarf any current system.
Potential Improvements and Research Directions
To move from a theoretical concept to a more practical proposition, several areas of research and development could be pursued:
Advanced Materials: Research into new materials with higher sensitivity to neutrino interactions could improve detection rates. Nanomaterials or quantum dots might offer new pathways to detect and harness the energy from neutrino interactions more efficiently.
Accelerator Technology: Developing more compact and efficient accelerators would reduce the initial and operational costs of generating high-intensity neutrino beams. Using new acceleration techniques, such as plasma wakefield acceleration, could significantly decrease the size and cost of accelerators.
Detector Technology: Improvements in photodetector efficiency and the development of new scintillating materials could enhance the signal-to-noise ratio in neutrino detectors. High-temperature superconductors could also be used to improve the efficiency of magnetic horns and focusing devices.
Energy Conversion Methods: Exploring direct conversion methods, where the kinetic energy of particles from neutrino interactions is directly converted into electricity, could bypass the inefficiencies of thermal conversion systems. Research into piezoelectric materials or other direct conversion technologies could be key.
Conceptual Experiment to Demonstrate Viability
To demonstrate the viability of neutrino power transmission, even at a very small scale, a conceptual experiment could be set up as follows:
Experimental Setup
Small-Scale Accelerator: Use a small-scale proton accelerator to generate a neutrino beam. For experimental purposes, this could be a linear accelerator used in many research labs, capable of accelerating protons to a few hundred MeV.
Miniature Target and Decay Tunnel: Design a compact target and a short decay tunnel to produce and focus neutrinos. This setup will test the beam production and initial focusing systems.
Small Detector: Construct a small-scale neutrino detector, possibly using a few tons of liquid scintillator or water, equipped with sensitive photodetectors. This detector will test the feasibility of detecting focused neutrino beams at short distances.
Measurement and Analysis: Measure the rate of neutrino interactions and the energy deposited in the detector. Compare this to the expected values based on the beam properties and detector design.
Steps to Conduct the Experiment
Calibrate the Accelerator and Beamline: Ensure the proton beam is correctly tuned and the target is accurately positioned to maximize pion and kaon production.
Operate the Decay Tunnel and Focusing System: Run tests to optimize the magnetic focusing horns and maximize the neutrino beam coherence.
Run the Detector: Collect data from the neutrino interactions, focusing on capturing the rare events and distinguishing them from background noise.
Data Analysis: Analyze the collected data to determine the neutrino flux and interaction rate, and compare these to
theoretical predictions to validate the setup.
Optimization: Based on initial results, adjust the beam energy, focusing systems, and detector configurations to improve interaction rates and signal clarity.
Example Calculation for a Proof-of-Concept Experiment
To put the above experimental setup into a more quantitative framework, here's a simplified example calculation:
Assumptions and Parameters
Proton Beam Energy: 500 MeV (which is within the capability of many smaller particle accelerators).
Number of Protons per Second ((N_p)): (1 \times 10^{13}) protons/second (a relatively low intensity to ensure safe operations for a proof-of-concept).
Target Efficiency: Assume 20% of the protons produce pions or kaons that decay into neutrinos.
Neutrino Energy ((E_\nu)): Approximately 30% of the pion or kaon energy, so around 150 MeV per neutrino.
Distance to Detector ((D)): 100 meters (to stay within a compact experimental facility).
Detector Mass: 10 tons of water (equivalent to (10^4) kg, or about (6 \times 10^{31}) protons assuming 2 protons per water molecule).
Neutrino Interaction Cross-Section ((\sigma)): Approximately (10^{-38} , \text{m}^2) (typical for neutrinos at this energy).
Neutrino Detection Efficiency: Assume 50% due to detector design and quantum efficiency of photodetectors.
Neutrino Production
Pions/Kaons Produced: [ N_{\text{pions/kaons}} = N_p \times 0.2 = 2 \times 10^{12} \text{ per second} ]
Neutrinos Produced: [ N_\nu = N_{\text{pions/kaons}} = 2 \times 10^{12} \text{ neutrinos per second} ]
Neutrino Flux at the Detector
Given the neutrinos spread out over a sphere: [ \text{Flux} = \frac{N_\nu}{4 \pi D^2} = \frac{2 \times 10^{12}}{4 \pi (100)^2} , \text{neutrinos/m}^2/\text{s} ] [ \text{Flux} \approx 1.6 \times 10^7 , \text{neutrinos/m}^2/\text{s} ]
Expected Interaction Rate in the Detector
Number of Target Nuclei ((N_t)) in the detector: [ N_t = 6 \times 10^{31} ]
Interactions per Second: [ R = \text{Flux} \times N_t \times \sigma \times \text{Efficiency} ] [ R = 1.6 \times 10^7 \times 6 \times 10^{31} \times 10^{-38} \times 0.5 ] [ R \approx 48 , \text{interactions/second} ]
Energy Deposited
Energy per Interaction: Assuming each neutrino interaction deposits roughly its full energy (150 MeV, or (150 \times 1.6 \times 10^{-13}) J): [ E_d = 150 \times 1.6 \times 10^{-13} , \text{J} = 2.4 \times 10^{-11} , \text{J} ]
Total Power: [ P = R \times E_d ] [ P = 48 \times 2.4 \times 10^{-11} , \text{J/s} ] [ P \approx 1.15 \times 10^{-9} , \text{W} ]
So, the power deposited in the detector from neutrino interactions would be about (1.15 \times 10^{-9}) watts.
Challenges and Improvements for Scaling Up
While the proof-of-concept might demonstrate the fundamental principles, scaling this up to transmit even a single watt of power, let alone megawatts, highlights the significant challenges:
Increased Beam Intensity: To increase the power output, the intensity of the proton beam and the efficiency of pion/kaon production must be dramatically increased. For high power levels, this would require a much higher energy and intensity accelerator, larger and more efficient targets, and more sophisticated focusing systems.
Larger Detector: The detector would need to be massively scaled
up in size. To detect enough neutrinos to convert to a practical amount of power, we're talking about scaling from a 10-ton detector to potentially tens of thousands of tons or more, similar to the scale of detectors used in major neutrino experiments like Super-Kamiokande in Japan.
Improved Detection and Conversion Efficiency: To realistically convert the interactions into usable power, the efficiency of both the detection and the subsequent energy conversion process needs to be near-perfect, which is far beyond current capabilities.
Steps to Scale Up the Experiment
To transition from the initial proof-of-concept to a more substantial demonstration and eventually to a practical application, several steps and advancements are necessary:
Enhanced Accelerator Performance:
Upgrade to Higher Energies: Move from a 500 MeV system to several GeV or even higher, as higher energy neutrinos can penetrate further and have a higher probability of interaction.
Increase Beam Current: Amplify the proton beam current to increase the number of neutrinos generated, aiming for a beam power in the range of hundreds of megawatts to gigawatts.
Optimized Target and Decay Tunnel:
Target Material and Design: Use advanced materials that can withstand the intense bombardment of protons and optimize the geometry for maximum pion and kaon production.
Magnetic Focusing: Refine the magnetic horns and other focusing devices to maximize the collimation and directionality of the produced neutrinos, minimizing spread and loss.
Massive Scale Detector:
Detector Volume: Scale the detector up to the kiloton or even megaton range, using water, liquid scintillator, or other materials that provide a large number of target nuclei.
Advanced Photodetectors: Deploy tens of thousands of high-efficiency photodetectors to capture as much of the light from interactions as possible.
High-Efficiency Energy Conversion:
Direct Conversion Technologies: Research and develop technologies that can convert the kinetic energy from particle reactions directly into electrical energy with minimal loss.
Thermodynamic Cycles: If using heat conversion, optimize the thermodynamic cycle (such as using supercritical CO2 turbines) to maximize the efficiency of converting heat into electricity.
Integration and Synchronization:
Data Acquisition and Processing: Handle the vast amounts of data from the detector with real-time processing to identify and quantify neutrino events.
Synchronization: Ensure precise timing between the neutrino production at the accelerator and the detection events to accurately attribute interactions to the beam.
Realistic Projections and Innovations Required
Considering the stark difference between the power levels in the initial experiment and the target power levels, let's outline the innovations and breakthroughs needed:
Neutrino Production and Beam Focus: To transmit appreciable power via neutrinos, the beam must be incredibly intense and well-focused. Innovations might include using plasma wakefield acceleration for more compact accelerators or novel superconducting materials for more efficient and powerful magnetic focusing.
Cross-Section Enhancement: While we can't change the fundamental cross-section of neutrino interactions, we can increase the effective cross-section by using quantum resonance effects or other advanced physics concepts currently in theoretical stages.
Breakthrough in Detection: Moving beyond conventional photodetection, using quantum coherent technologies or metamaterials could enhance the interaction rate detectable by the system.
Scalable and Safe Operation: As the system scales, ensuring safety and managing the high-energy particles and radiation produced will require advanced shielding and remote handling technologies.
Example of a Scaled Concept
To visualize what a scaled-up neutrino power transmission system might look like, consider the following:
Accelerator: A 10 GeV proton accelerator, with a beam power of 1 GW, producing a focused neutrino beam through a 1 km decay tunnel.
Neutrino Beam: A beam with a diameter of around 10 meters at production, focused down to a few meters at the detector site several kilometers away.
Detector: A 100 kiloton water Cherenkov or liquid scintillator detector, buried deep underground to minimize cosmic ray backgrounds, equipped with around 100,000 high-efficiency photodetectors.
Power Output: Assuming we could improve the overall system efficiency to even 0.1% (a huge leap from current capabilities), the output power could be: [ P_{\text{output}} = 1\text{ GW} \times 0.001 = 1\text{ MW} ]
This setup, while still futuristic, illustrates the scale and type of development needed to make neutrino power transmission a feasible alternative to current technologies.
Conclusion
While the concept of using neutrinos to transmit power is fascinating and could overcome many limitations of current power transmission infrastructure, the path from theory to practical application is long and filled with significant hurdels.
#Neutrino Energy Transmission#Particle Physics#Neutrino Beam#Neutrino Detector#High-Energy Physics#Particle Accelerators#Neutrino Interaction#Energy Conversion#Direct Energy Conversion#High-Voltage Direct Current (HVDC)#Experimental Physics#Quantum Materials#Nanotechnology#Photodetectors#Thermoelectric Generators#Superfluid Helium#Quantum Dots#Plasma Wakefield Acceleration#Magnetic Focusing Horns#Cherenkov Radiation#Scintillation Light#Silicon Photomultipliers (SiPMs)#Photomultiplier Tubes (PMTs)#Particle Beam Technology#Advanced Material Science#Cost-Effectiveness in Energy Transmission#Environmental Impact of Energy Transmission#Scalability of Energy Systems#Neutrino Physics#Super-Kamiokande
0 notes
Text
https://esp-safety.in/product/particle-detection/subsea/echo-uw
In the realm of modern scientific exploration, the quest to uncover the mysteries of the universe hinges on the development of advanced technologies. Among these, particle detectors stand as indispensable tools, allowing scientists to observe and analyze the fundamental building blocks of matter. At the forefront of this technological frontier is QuantumVision, a leading Particle Detector manufacturer dedicated to providing state-of-the-art particle detector solutions for cutting-edge research.
0 notes
Text
I like the name "Oh my god particle."
0 notes
Text
Enhancing Subsea Pipeline Integrity with ESP Asset Integrity's Subsea PIG Detector Sensors
The Subsea PIG Detector sensors by ESP Asset Integrity are innovative devices designed to detect and monitor Pipeline Inspection Gauges (PIGs) in subsea environments. These advanced sensors are crucial for ensuring the integrity and safety of underwater pipelines. They utilize cutting-edge technology to accurately locate and track the movement of PIGs within the pipelines, allowing for timely maintenance and preventing potential damages or leakages. ESP Asset Integrity's Subsea PIG Detector sensors are a reliable and essential tool for the oil and gas industry, providing valuable data and insights to enhance the overall efficiency and longevity of subsea pipeline operations. Visit our website to know more.
#Subsea PIG Detector sensors#Acoustic Particle Monitoring#corrosion detector#acoustic sand detector#PIG Detectors
0 notes
Text
clear pro of being surrounded by scientists is that nobody gives a fuck what you look like. i saw a guy walking around barefoot yesterday. i could probably go to work looking like i just fell out of bed and nobody would even look at me weird.
#tütensuppe#next time im getting a haircut ill dye it again#on thursday i was in a meeting where someone presented conference results#something something scientist sport but the detector called BREAD amused me a LOT#its the contrast between the serious looking presentation slides and then the thing is just called BREAD#aside from that it feels like everyone is trying to get to space first#i think if they collaborated a bit more rather than building the same thing in different places theyd find the#mystery particle faster
0 notes
Text
⠂⠄⠄⠂⠁⠁⠂⠄⠄⠂⠁⠁⠂⠄⠄⠂ ⠂⠄⠄⠂☆
the double slit experiment and shifting



imagine a wall with a single vertical slit in it and a beam of light pointed at it. looking on the other side of that wall, you would see a single line of light from where it passed through the slit.
now, imagine there were two slits in that wall. what would you expect to see on the other side? well, you wouldn’t see two strips of light to match the slits. instead, the light behaves like waves. like if you were to drop two rocks in water and watch the waves they create intersect and ripple off each other.
the double slit experiment was to prove that light behaves less as particles, and more as a wave.
now— the interesting part.
even when firing electrons one by one through the slits, the wave pattern was still formed. curious about how this happens, a detector is placed by the slits to find out which slit an electron passes through first.
except, with the detector there, the electrons suddenly start acting as particles and not waves. they now produce two strips on the back wall. the act of being observed changed how the electrons behaved.
the most popular theory for this phenomenon is called the Copenhagen interpretation, but it’s not the one i wanted to highlight.
the collapse of the electrons from waves to particles could happen anywhere from the detector measuring them to the mind processing the information. the von Neumann-Wigner interpretation argued that the collapse happens when a consciousness interprets the measurement, and that without the consciousness, it would just be a wave of probabilities.
the Many-Worlds interpretation argued that there is never any collapse at all, but that all possible measurement outcomes exist in different worlds. while we may be seeing one measurement in our world, there is another world where there is a different measurement outcome, or even no measurement at all.
so, that’s one interpretation about consciousness determining reality, and another about the existence of multiple worlds— sound familiar?
the two leading theories for shifting are the consciousness theory and the multiverse theory. the first one suggesting that consciousness creates reality, and the second proposing that there are infinite realities. ideas that are absolutely identical to the previously mentioned interpretations.
it’s not like this proves anything, but i find it interesting that shifting-related concepts can be found in places like quantum mechanics. it makes me hopeful that, one day, shifting will be accepted, or at least considered, by the general public.
⠂⠄⠄⠂⠁⠁⠂⠄⠄⠂⠁⠁⠂⠄⠄⠂ ⠂⠄⠄⠂☆
i really really hope this is digestible, but sorry if it isn’t, i’m assuming most people don’t go on tumblr expecting to read an essay on quantum mechanics lol
#shifting#shiftblr#shifting realities#reality shifting#reality shift#desired reality#shifting community#shifters#anti shifters dni#shiftingrealities#shifting blog#shifting reality#shifter#shifting antis dni#shifting motivation#shifting consciousness
88 notes
·
View notes
Text

This is Professor Agnieszka Zalewska.
She specialises in particle physics. She received her doctorate in 1975 from the Jagellonian University in Krakow, Poland, for work carried out on bubble chamber data from an experiment at CERN. Later, she worked on the DELPHI experiment at CERN's Large Electron Positron collider, LEP, where she played an important role in the development of silicon tracking detectors. Since 2000, she has been involved with neutrino physics through the ICARUS experiment at Italy's Gran Sasso National Laboratory, which studies a neutrino beam sent through the Earth from CERN, and has also been involved with feasibility studies for an underground laboratory in Poland.
She was the first woman to be elected President of CERN Council (2012). She was also the first scientist from Central and Eastern Europe.
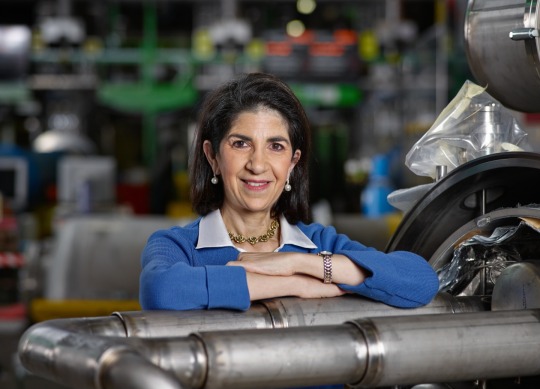
Also, let's not forget that today, CERN is led by a woman. Professor Fabiola Gianotti became the first woman elected Director General of CERN (2016). She was renewed for the second term of office in 2021.
#I saw something today and had to do this#it's always good to spread my agenda#physics#science#women
678 notes
·
View notes